What are we talking about?
Before entering the subject of subsidence, we must distinguish it from breakwater destruction by wave action. The latter yields spreading of materials on the sea floor resulting in a complete destruction of the breakwater superstructure which can then barely be recognised as such under water. This is not (or less) the case with subsidence yielding a vertical movement, possibly combined with tilting, of the structures.
Subsidence must also be distinguished from local scour near the toe of the structure when breaking of waves coming in obliquely induce a longshore current that might yield erosion of the sandy bed in front of the structure. This may undermine the offshore toe of the structure and cause tumbling of the large capping blocks towards the sea, but not a uniform subsidence of the whole structure.
Repeated storms have sometimes been put forward as a possible explanation for the breakwater subsidence due to wave-induced liquefaction. From a hydraulic point of view, we must visualise a wave travelling towards the coast with a crest parallel to the breakwater. This wave is reflected by the offshore side of the breakwater, inducing a nearly double wave height in front of it. Large waves might indeed induce local liquefaction of the sandy seabed on the offshore side of the breakwater (Zen, 1990 & 1991). This induces a subsidence larger at that side than at the inner side of the breakwater and tumbling of large concrete blocks towards the offshore side would be observed rather than a uniform vertical subsidence.
A different mechanism is that of wave-induced compaction of the sub-soil underneath the structure. Before breakwaters are built, the seabed often consists of more or less loosely packed sand provided by longshore sediment transport. Adding the weight of the breakwaters and subjecting them to vibrations due to wave action and to seismic action, will induce compaction of the sub-soil. In addition, consolidation of clayey materials (if any) and long-term deformation called creep may also play a role in coastal areas at a centennial or millennial time-scale. Modern engineers always dredge away these layers of loosely packed and clayey materials before building any structure, but ancient builders probably did not, because the required heavy-duty dredging equipment did not exist.
Because of the large waves acting on the outer side of the breakwater, a cyclic hydraulic gradient is generated between both sides of the breakwater. This induces a strong flow inside the rubble mound of the breakwater or at the interface between the large concrete blocks and the unprotected sandy seabed. In order to avoid irreversible problems with the foundation of large marine structures due to piping and undermining, a foundation layer consisting of a “granular filter” must be installed in accordance with strict requirements (de Graauw, 1984). As a matter of fact, foundation layers consisting of fine granular material (say 2 to 50 mm) placed underneath large blocks made of Roman concrete are an essential part of their foundation, but they have not been mentioned by excavators so far, except in Caesarea Maritima and Athlit, where a layer of cobbles (probably ship ballast) was found (Votruba, 2007, Haggi, 2005). Only one other case has been recently noted in Fos where pillars made of ashlar were “laid on a level of coarse sand mixed with fragments of ceramics. Below this level, finer sand is largely mingled with dead posidonia” (Fontaine, 2021), but it is suspected that this perfect filter layer with Posidonia Oceanica was not entirely intentional … Another case is reported by Marty (2016), also at Fos, where a layer of Posidonia was deliberately used as a filter.
Other explanations include earthquakes inducing tsunamis. The tsunami wave(s) first encounters the outer face of the breakwater, where part of its energy is reflected back to the open sea. At this stage, the tsunami might push large blocks of Roman concrete placed on top of the breakwater into the port, rather than generating a uniform vertical subsidence. Then, depending on the size of the tsunami, a substantial part of the energy would overflow the breakwater and submerge the whole harbour area, taking away all loose blocks, pavements, warehouses, ships, etc. The tsunami wave would then enter the city and would finally flow back to sea, taking much waste into the harbour, but it has been shown elsewhere that it can be really hard to distinguish ancient tsunami deposits from other deposits.
Earthquake-generated liquefaction is a convenient explanation for subsidence as it is likely to affect large areas covered with cohesionless water-saturated sand. It was probably mentioned by Aelius Aristides (Oration 19) who witnessed the 178 AD earthquake in Smyrna: “some of the temples have fallen, some sunk beneath the ground”. It is probably a major explanation for the subsidence observed at Caesarea Maritima.
The potential for liquefaction depends on the sub-soil properties (Idriss & Boulanger, 2008 ; Hettler, 2014): sand must be loosely packed (less than 70% relative density) and may include a small fraction of fine silts or clay, so-called “silty sand” (less than 20% with a diameter below 74 microns). Longshore transport of sediment often provides this kind of sand in the nearshore area down to a water depth of ca. 10 m.
During an earthquake, sand with a large porosity (say 40% for a loose packing) will tend to re-arrange its packing and reduce its porosity (to say 30% for a dense packing). This will require some pore water to seep out of the sub-soil, but that flow may be delayed by low-permeability materials. Any load resting on this sub-soil would then be floating on water instead of resting on a solid skeleton of sand grains, and as water would gradually flow out, the load would gradually sink into the sub-soil until it would rest on the re-arranged sand skeleton. This liquefaction process is a short term one occurring within minutes during and shortly after the earthquake. This is of course an idealised and simplified scenario, and many complications may occur in reality with superimposed layers of various materials, including impermeable layers, etc.
According to this process, liquefaction can only occur once in a given area.
Last but not least, we mention tectonic subsidence. This involves crustal movements of the earth which may be horizontal, vertical, or combined. This also involves faults along which such crustal movements appear during earthquakes. It must be reminded that a meters-high subsidence due to tectonic movements is a major and catastrophic event with many casualties that is usually reported even in ancient literature.
Where did we observe subsidence of coastal structures?
Now we have a better understanding of the phenomena involved, let’s have a closer look at places where subsidence was observed (Flemming, 1978; Auriemma, 2009; Pavlopoulos, 2011; Kolaiti, 2023).
Let’s make a few preliminary notes on the available data:
- Subsidence (and uplift) may be a continuous process (e.g., consolidation) or a sudden process (e.g., liquefaction). Average rates of subsidence in mm/year make sense only in case of continuous processes.
- It is very difficult to separate eustatic Sea Level Rise (SLR) and subsidence of the earth’s crust. The Relative Sea Level Rise (RSLR or Submergence) of around 0.25 mm/year over the past 2000 years may be due for a large part (maybe even totally) to crustal subsidence. Hence, places that were submerged more 0.5 m over the past 2000 years must have been subject to some kind of subsidence (as Submergence = Subsidence + SLR).
- In addition, RSLR was around 0.7 mm/year over the 5000 years before that. Therefore, we selected data with an age less than 5000 years (from now to 3000 BC).
- If the eustatic SLR amounted to ca. 0.5 m in the past 2000 years, any uplift larger than 0.5 m will be visible on land without underwater exploration.
Sites with more than 1 m submergence in 2000 years (0.50 mm/yr) were selected from our data base, yielding 344 sites (including Atlantis!).
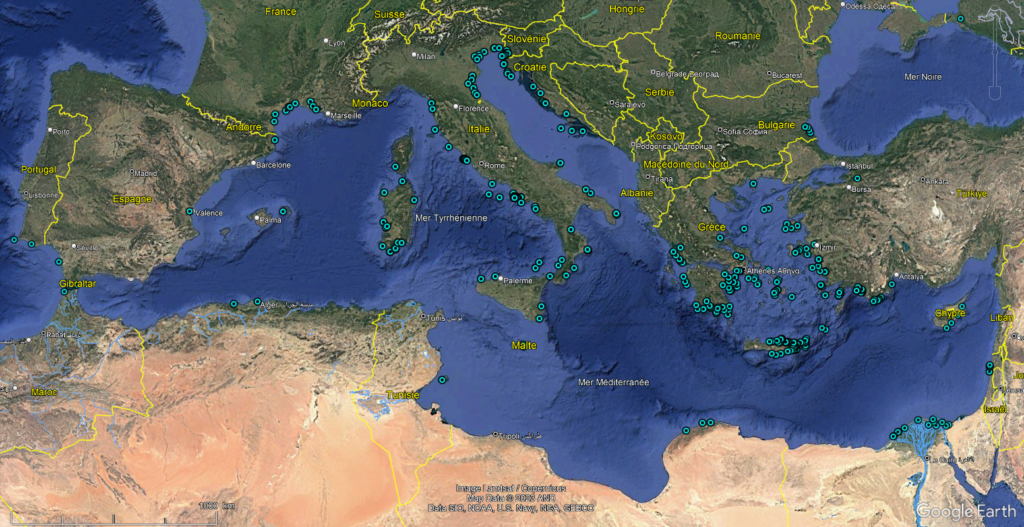
Submerged sites are found in the Rhône delta, the Tyrrhenian coast, the bay of Naples, the Pô delta (Ravenna and Aquileia), several sites around the Peloponnesus and on Paros Island in the Cyclades, eastern Crete, many places on the SW Turkish coast between Izmir and Antalya, southern Cyprus, the Nile delta (Thonis-Herakleion, Alexandria), Cyrenaica (Apollonia).
Port structures located on loosely packed sands provided by longshore sediment transport may be subject to liquefaction during earthquakes, inducing a general subsidence of the port. Sites in deltas are well-known for subsidence which is usually due to compaction of underlayers that are loaded with new sediment brought by the river(s). In addition, consolidation may occur if these underlayers contain clayey materials. Sites in rocky areas may be subjected to crustal movements linked to seismic activity, like around the Aegean Sea. The bay of Naples is a particular case subjected to so-called ‘bradyseism’ linked to volcanic activity and resulting in alternating uplift and subsidence.
It is usually quite difficult to go into further detailed explanations of subsidence of coastal sites because many geological and geotechnical aspects are involved. An example is provided in this volume for Caesarea Maritima. Another case is Thonis-Heracleion (Brocard, 2024).
A similar exercise showed 80 sites uplifted in the last 2000 years. Some of them are located in Calabria (Ferranti, 2017), northern Peloponnesus, Samos (Stiros, 2000), Rhodes (Triantafyllou, 2022), western Cilicia (Liberatore, 2023), northern Levant (Sivan, 2010), but most are located in western Crete as a result of the tilting of the island during the 21/7/365 AD earthquake. During this event, the ancient port of Phalasarna and the ancient Kissamos breakwater were uplifted by ca. 6 m. A similar event occurred on the Noto Peninsula (Japan) on 1/1/2024 when the coastline was uplifted by 4 m, leaving Monzenmachi Kuroshima fishing harbour completely dry.
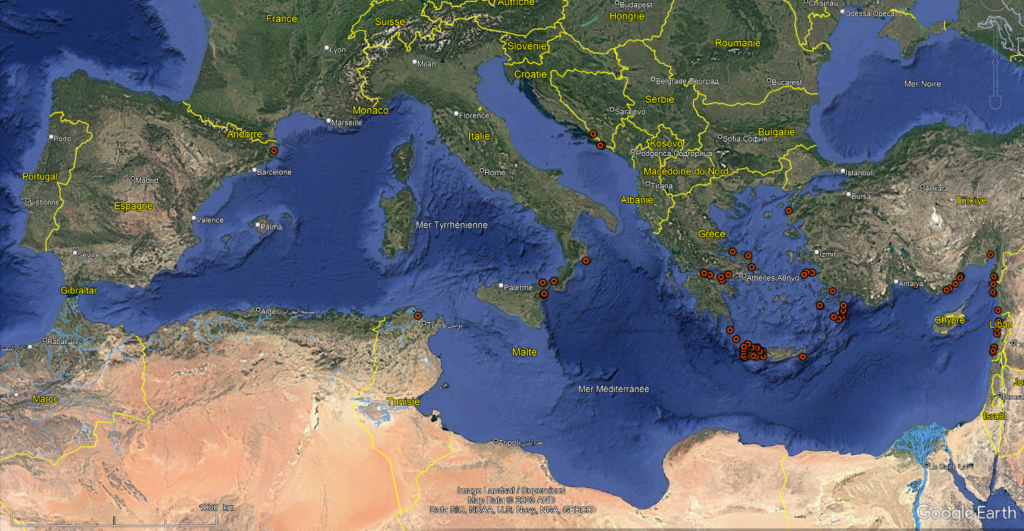
References
AURIEMMA, R. & SOLINAS, E., 2009, “Archaeological remains as sea level change markers: A review”, Quaternary International 206 (2009), (p 134-146).
BROCARD, G., GOIRAN, J-P, ROBINSON, D., “Fate of two cities built on sinking ground: slow and fast submergence at Thônis – Heracleion and Canopus, Nile River Delta, Egypt”,
https://www.researchgate.net/publication/386134754
DE GRAAUW, A., 1984, “Design criteria for granular filters”. J. Waterw., Port, Coast. and Ocean Eng., ASCE 110 (1984) 1. Delft Hydraulics Laboratory, Publication n° 287, (25 p).
FLEMMING, N., 1978, “Holocene Eustatic Changes and Coastal Tectonics in the Northeast Mediterranean: Implications for Models of Crustal Consumption”, Philosophical Transactions of the Royal Society of London. Series A, Mathematical and Physical Sciences, Vol. 289, No. 1362, (p 405-458).
FONTAINE, S., EL-AMOURI, M., MARTY, F., ROUSSE, C., 2021, “The Submerged Monumental Complex of the Roman Harbour of Fossae Marianae, Gulf of Fos, France – An overview of preliminary results”, in: Under the Mediterranean I. Studies in Maritime Archaeology, Sidestone Press, (p 181-194).
HAGGI, A., 2005, “Underwater excavation at the Phoenician harbor at Athlit, 2002 season”, R.I.M.S. News, report N° 31, Haifa.
HETTLER, A., 2014, “Recommendations on Excavations”, DGGT, Deutsche Gesellschaft für Geotechnik, Wiley, (286 p).
IDRISS, I., & BOULANGER, R., 2008 “Soil liquefaction during earthquakes”, Earthquake Engineering Research Institute, Oakland, CA, USA, (264 p).
KOLAITI, E., KIZILDAG, N., ÖZDAS, H., MOURTZAS, N., 2023, “Relative Sea-Level Changes in the Central Aegean from the Late Roman/Early Byzantine Period Onwards”, Recent Research on Environmental Earth Sciences, Geomorphology, Soil Science, Paleoclimate,and Karst, Proceedings of the 1st MedGU, Istanbul 2021 (Volume 4), Springer, (p 147-150).
LIBERATORE, M., et al., 2023, ‘’Vertical velocity fields along the Eastern Mediterranean coast as revealed by late Holocene sea-level markers’’, Earth-Science Reviews 234, Elsevier, (p 1-26).
MARTY, F., GUIBAL, F., HESNARD, A., “L’Estagnon : techniques de bonification d’une zone palustre au 1er s. ap. J.-C. à Fos-sur-Mer (Bouches-du-Rhône)”, Colloque “Les ports dans l’espace méditerranéen antique”, Montpellier 2014, (p 263-278).
PAVLOPOULOS, K., et al., 2011, “Vertical displacement trends in the Aegean coastal zone (NE Mediterranean) during the Holocene assessed by geo-archaeological data”, The Holocene, 22(6), (p 717-728).
SIVAN, D., et al., 2010, “What can a sessile mollusk tell about neotectonics?”, Earth and Planetary Science Letters, 2010, 296, (p 451-458).
STIROS, S., et al., 2000, “Seismic coastal uplift in a region of subsidence: Holocene raised shorelines of Samos Island, Aegean Sea, Greece”, Marine Geology 170, (p 41-58).
TRIANTAFYLLOU, I., 2022, “Historical co-seismic uplift rates in the eastern Hellenic subduction zone: the case of Rhodes Island”, Zeitschrift für Geomorphologie, Vol. 63/2–3, (p 201–217).
VOTRUBA, G., 2007, “Imported Building Materials of Sebastos Harbour, Israel”, International Journal of Nautical Archaeology, 2007, 36.2, (p 325-335).
ZEN, K., & YAMAZAKI, H., 1991, “Field observation and analysis of wave-induced liquefaction in seabed”, Soils and Foundations, Vol. 31, No.4, Japanese Society of Soil Mechanics and Foundation Engineering, (p 161-179).
ZEN, K., & YAMAZAKI, H., 1990, “Mechanism of wave-induced liquefaction and densification in seabed”, Soils and Foundations, Vol. 30, No. 4, Japanese Society of Soil Mechanics and Foundation Engineering, (p 90-104).